Exploring the Mechanisms of Neuronal Action Potential
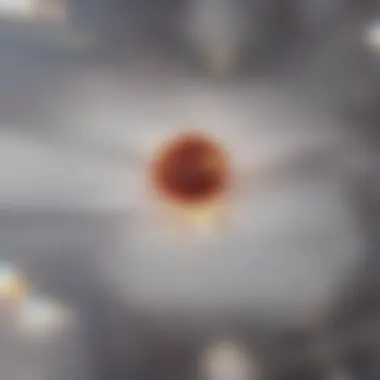
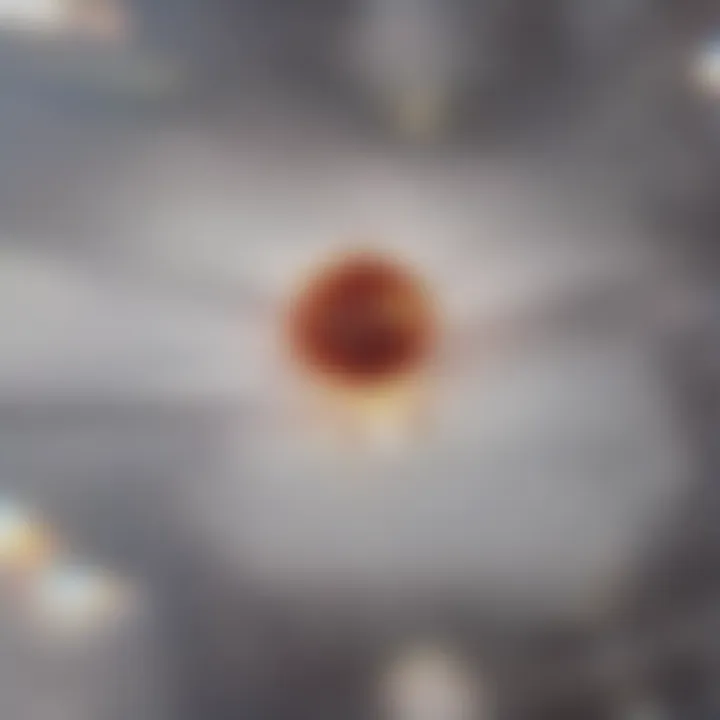
Intro
Understanding action potentials in neurons is essential for grasping how signals travel in the nervous system. This process is the basis of communication between nerve cells, influencing everything from reflexes to complex brain functions. The action potential is an electrical signal that, when properly understood, reveals much about neuronal behavior and its implications for both health and disease.
Background and Context
Overview of the Research Topic
The study of action potentials dates back to the early 20th century, when scientists began to explore how neurons generate and propagate electrical impulses. This understanding has evolved, enabling researchers to investigate various aspects of neuronal signaling. Action potentials underlie activities from simple movements to advanced cognitive functions, highlighting their significance in neuroscience.
Historical Significance
Historically, the work of pioneering scientists like Alan Hodgkin and Andrew Huxley in the 1950s provided a mathematical model for understanding how action potentials occur. Their research deservedly earned them the Nobel Prize in Physiology or Medicine in 1963. By dissecting the components involved, they laid the groundwork for modern neuroscience. Today, the implications of action potentials extend beyond basic research to clinical applications, influencing treatments for neurological disorders.
Key Findings and Discussion
Major Results of the Study
Recent studies have highlighted several factors that influence action potentials, such as ion channels' roles and synaptic interactions. For instance, sodium (Na+) and potassium (K+) ions are essential for the initiation and propagation of action potentials. Disruptions in these ionic movements can lead to conditions like epilepsy or multiple sclerosis.
Detailed Analysis of Findings
The action potential process can be broken down into distinct phases:
- Resting Phase: Neurons maintain a stable, negative interior compared to the outside.
- Depolarization: A stimulus triggers an influx of sodium ions, causing a rapid increase in membrane potential.
- Repolarization: Following the peak, potassium ions exit the cell to restore the negative interior.
- Hyperpolarization: Often, the membrane potential becomes even more negative than the resting state, temporarily inhibiting further action potentials.
"The action potential is a fundamental aspect of neuronal communication, with each phase playing a critical role in ensuring effective signal transmission."
This sequence reveals how neurons communicate with precision and speed. A thorough understanding of action potentials enhances our comprehension of both normal physiology and the pathophysiology of various neurological conditions. As neuroscience continues to advance, action potentials will remain a cornerstone of research efforts.
Overall, exploring the mechanisms of action potential generation and propagation sheds light on both basic and clinical neuroscience. This knowledge is crucial for educators, students, researchers, and professionals aiming to deepen their understanding of neuronal function.
Preamble to Neuronal Action Potential
The concept of neuronal action potential serves as the foundation for understanding how neurons communicate and transmit information. It is essential to grasp the dynamics of action potentials to comprehend broader neurophysiological processes.
An action potential is essentially an electrical signal that travels along the axon of a neuron. This signal is critical for the transmission of information in the nervous system. Without action potentials, the intricate dance of communication within the brain would cease to function, leading to a breakdown in neurobiological processes that support thought, emotion, and movement. The process by which action potentials occur involves a series of complex mechanisms influenced by ionic movements across neuronal membranes, which creates the rapid changes in voltage that characterize action potentials.
In the context of this article, understanding action potentials is not only important for students and researchers in neuroscience but also for educators and healthcare professionals. The relevance of action potentials extends to the study of neurological disorders, where disruptions to these signaling mechanisms can lead to various pathological conditions. Being informed about action potentials provides insights into how treatments may be developed or how neurological phenomena can be addressed in clinical settings.
Overall, elucidating the principles of action potentials helps in grasping how neurons function collectively to form networks that facilitate the processing and integration of sensory information, thereby ensuring the body responds appropriately to both internal and external stimuli.
Definition of Action Potential
An action potential is defined as a sudden and rapid change in the electrical charge across a neuron's membrane. This process occurs when the neuron reaches a certain threshold of depolarization, leading to a cascade of ionic movements that generate a spike in electrical activity.
- Resting State: This is when the neuron is not sending signals; the inside of the cell is negatively charged compared to the outside.
- Threshold Reached: When a stimulus causes the cell membrane to depolarize to a critical level, the action potential is triggered.
- Rapid Depolarization: Voltage-gated sodium channels open, allowing sodium ions to flow into the cell, making it more positive.
- Repolarization: After reaching a peak voltage, sodium channels close, and potassium channels open, allowing potassium ions to exit the cell, restoring the negative internal charge.
- Hyperpolarization: The cell briefly becomes more negative than its resting potential.
Thus, the action potential can be seen as a wave of electrical charge that propagates down the axon, leading to synaptic transmission and communication with other neurons.
Significance in Neuroscience
The significance of action potentials in neuroscience cannot be overstated. They are fundamental to neuronal communication, allowing for rapid information transfer across vast networks of neurons. Understanding action potentials contributes to our knowledge of various critical processes:
- Neural Communication: Action potentials facilitate the connections between neurons, which is vital for reflexes, sensory processing, and cognitive functions.
- Synaptic Transmission: Action potentials influence neurotransmitter release at synapses, where they enable signals to pass from one neuron to another.
- Neurological Disorders: Many disorders, such as epilepsy and multiple sclerosis, relate to dysfunctional action potentials. Research into these disorders often centers around how electrical signaling can go awry.
The ongoing research into action potential dynamics has the potential to lead to breakthroughs in treatments for neurological conditions. Insights generated from understanding how action potentials function can inform the development of pharmacological therapies targeted at specific ionic channels and mechanisms.
"Understanding action potentials provides the cornerstone for grasping the complex interactions that govern neuronal behavior, influencing everything from reflex actions to intricate cognitive tasks."
Neuronal Structure and Function
Understanding the structure and function of neurons is critical to grasping the concept of action potentials. Neurons are the fundamental units of the nervous system. Their specialized structure allows them to carry out essential tasks such as transmitting signals across the body. By examining various components of a neuron, one can appreciate how their unique features contribute to the overall aim of conveying information efficiently.
Components of a Neuron
Cell Body
The cell body, or soma, is a crucial element of a neuron. It contains the nucleus, which houses genetic material and regulates cellular activities. The key characteristic of the cell body is its role in integrating signals received from dendrites and generating an action potential if the threshold is met. This function is particularly beneficial as it serves as the control center, ensuring that the neuron can process and respond to stimuli effectively.
The unique feature of the cell body is its clustering of organelles. Organelles such as mitochondria, rough endoplasmic reticulum, and Golgi apparatus are vital. They ensure proper cellular metabolism and support the neuron's high energy demands. However, if the cell body sustains extensive damage, it can lead to neuronal death, posing potential disadvantages in the context of neurodegeneration.
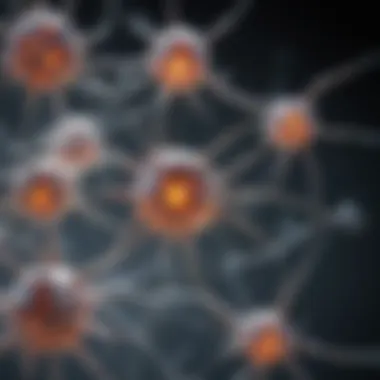
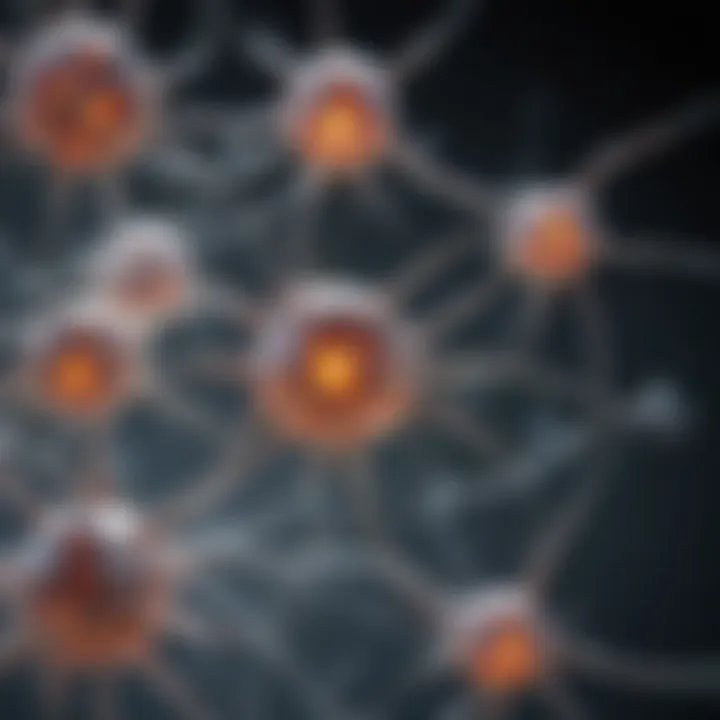
Dendrites
Dendrites are branch-like structures that extend from the cell body. They play a significant role in receiving synaptic inputs from other neurons, thus contributing to the neuron's overall functioning. The key characteristic of dendrites is their extensive surface area, which allows for the receipt of numerous signals simultaneously. This property makes them a crucial component in enhancing neural communication.
One unique aspect of dendrites is their ability to undergo structural changes in response to learning and experience, a process known as synaptic plasticity. This adaptability is advantageous as it enables the nervous system to modify responses based on new information. However, excessive or improper signaling can lead to excitotoxicity, a disadvantage related to dendritic function, which can be harmful to the neuron.
Axon
The axon is another central component of a neuron, mainly responsible for transmitting action potentials away from the cell body to other neurons or muscles. The length and diameter of the axon can vary, and a key characteristic is its ability to facilitate rapid conduction of electrical signals. This speed is paramount for effective communication within the nervous system.
A distinctive feature of axons is the presence of myelin sheath, which insulates parts of the axon. This insulation aids in the efficient transmission of action potentials. Additionally, the axon terminal plays an essential role in the release of neurotransmitters, making it vital for synaptic communication. However, if the axon is damaged, recovery can be limited, leading to challenges in restoring function in the entire nerve pathway.
Role of Myelin Sheath
The myelin sheath surrounds many axons, serving to expedite signal transmission. It is composed of lipid-rich substances that insulate the axon, reducing the loss of electrical signal strength. This role is critical, as without myelin, conducting electrical impulses would be slower, impairing communication between neurons. The key characteristic of myelin is not just speed; it also protects axons from potential damage and influences their overall health.
A unique aspect of myelin is that it forms interruptions, called nodes of Ranvier, along the axon. These nodes are where action potentials can jump, resulting in enhanced signal transmission speed, referred to as saltatory conduction. This feature is advantageous for maintaining rapid communication across long distances in the nervous system.
In summary, the structure of neurons—including the cell body, dendrites, axon, and myelin sheath—enables their intricate functions. Each component is tailored to ensure that neurons can fulfill their role effectively in action potential generation and propagation, contributing to the complex yet efficient workings of the nervous system.
Electrophysiology of Neurons
Electrophysiology is a crucial aspect of understanding neurons, as it provides insights into the electrical properties that mediate neuronal signaling. At its core, electrophysiology examines how neurons communicate through electrical impulses, primarily focusing on action potentials. By studying these electrical changes, we gain a better grasp of how information is processed in the nervous system.
The significance of electrophysiology lies in its ability to quantify and analyze the differences in voltage across the neuronal membrane. These voltage changes are fundamental to how neurons integrate and transmit signals.
Resting Membrane Potential
The resting membrane potential is the designator of the neuron's readiness to fire. Typically, neurons maintain a resting membrane potential of about -70mV. This negative value is primarily due to the uneven distribution of ions across the cell membrane, particularly sodium and potassium ions. When at rest, the cell membrane is more permeable to potassium ions than sodium ions, leading to a net negative charge inside the neuron. Such an electrical state is essential for the continuation of neuronal activity.
The resting membrane potential acts as the baseline from which action potentials are generated. Hence, it is a pivotal aspect in the overall functioning of neurons, as any significant deviation can influence subsequent signaling.
Ion Channels and Pumps
Ion channels and pumps play a fundamental role in establishing and maintaining the resting membrane potential. Each type of channel or pump has specific functions that contribute to the overall dynamics of neuronal excitability.
Voltage-Gated Channels
Voltage-gated channels provide a pathway for ions to flow across the neuronal membrane during changes in membrane potential. Specifically, these channels are sensitive to voltage changes across the membrane, allowing them to open or close in response to depolarization or repolarization of the neuron. This characteristic makes them crucial for the initiation and propagation of action potentials.
One key characteristic of voltage-gated channels is their swift activation and inactivation. This rapid response is advantageous, ensuring that action potentials are generated precisely when needed. However, these channels can be subject to inactivation, which may pose limitations in high-frequency firing.
Leak Channels
Leak channels allow for the passive movement of certain ions, providing a steady ionic current that helps sustain the resting membrane potential. They are not gated and therefore do not require a change in voltage to allow ions to pass through. The continual flow of ions through these channels is essential for maintaining the baseline state of the neuron.
The unique feature of leak channels is their constant contribution to the resting potential, allowing for homeostasis within the neuron. However, they are relatively non-specific, which may affect the precise control of the ion concentrations over time.
Sodium-Potassium Pump
The sodium-potassium pump is an essential active transport mechanism that helps maintain the resting membrane potential and overall cellular homeostasis. This pump works by using ATP to transport sodium ions out of the neuron while bringing potassium ions in, thus counteracting the natural diffusion of these ions.
A key characteristic of the sodium-potassium pump is its critical role in sustaining the ion gradients essential for action potential generation. The pump’s activity is vital for re-establishing the resting membrane potential after an action potential has occurred. One disadvantage, however, is that it requires energy, making it a metabolically expensive process.
The balance maintained by ion channels and pumps is vital, as any disruption can lead to altered neuronal excitability and influence overall neural function.
Understanding these key components of ion channels and pumps not only enriches our grasp on action potentials but also highlights the intricate mechanisms that underlie neuronal performance. Each feature contributes uniquely to the cell’s excitability and has implications for both normal and pathological states in neural systems.
Phases of Action Potential
The phases of action potential are essential for understanding how neurons transmit signals. They describe specific stages through which a neuron passes when it fires. The process is not only fundamental for neuronal communication but also critical for many physiological functions. Each phase has distinct characteristics and mechanisms that allow for the precise relay of information. Through the different phases—depolarization, repolarization, and hyperpolarization—neurons efficiently communicate messages across the nervous system. This understanding is vital for professionals in neuroscience and related fields.
Depolarization Phase
During the depolarization phase, the neuron's membrane potential rapidly shifts. Typically, the membrane potential starts at around -70 millivolts in its resting state. When a stimulus reaches the neuron and is strong enough to trigger an action potential, voltage-gated sodium channels open. As sodium ions rush into the neuron, the inside of the cell becomes more positive. This surge of sodium ions causes the membrane potential to rise towards the threshold level of approximately -55 millivolts.
This phase is crucial because it determines whether an action potential will occur. If the stimulus is sufficient, the rapid influx of sodium ions will lead to a significant potential change. This initial phase is a critical link in the chain of events that allows for messages to be sent throughout the nervous system.
Repolarization Phase
The repolarization phase follows depolarization and is characterized by the closing of sodium channels and the opening of potassium channels. As sodium influx subsides, potassium ions exit the neuron. This movement of potassium ions out of the cell begins to restore the internal environment to its original negative state. The membrane potential drops back towards the resting level.
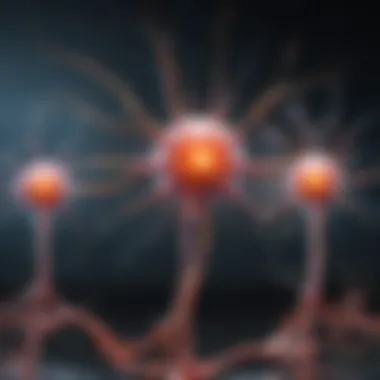
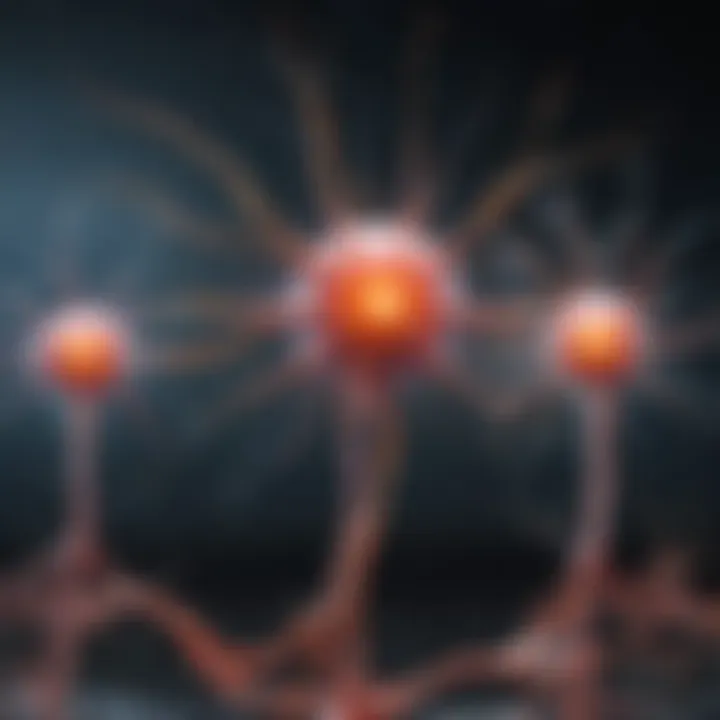
This phase is important because it allows the neuron to return to its baseline state, preparing it for the next action potential. The function of repolarization ensures that the action potential travels in the correct direction along the neuron. Without this phase, continuous firing could be problematic, leading to uncontrolled excitability of neurons.
Hyperpolarization Phase
Hyperpolarization occurs right after repolarization. During this phase, the membrane potential becomes even more negative than the resting potential, often reaching around -80 millivolts. This happens because potassium channels remain open longer than needed, allowing more potassium to exit the cell. This makes it harder for the neuron to generate another action potential immediately, which is referred to as the refractory period.
Hyperpolarization plays a critical role in preventing excessive firing of action potentials. This phase ensures that neurons communicate effectively and at appropriate rates. It contributes to the overall regulation of neuronal excitability, providing a mechanism that helps maintain balance within neural circuits.
Proper understanding of each phase is crucial to grasp the entire process of neuronal signaling.
The phases of action potential—depolarization, repolarization, and hyperpolarization—work together seamlessly to facilitate neuronal communication. Each stage is critical in ensuring the correct relay of signals and maintaining overall neuromuscular health. Without proper functioning of these phases, various neurological disorders can arise.
Threshold Potential
Threshold potential is a critical concept in the understanding of action potentials within neurons. It refers to the specific level of membrane depolarization that must be reached for an action potential to be initiated. This phenomenon is integral to neuronal communication, signaling the transition from the resting state of a neuron to the rapid firing of an action potential, thus facilitating the transmission of information across the nervous system.
Definition and Importance
The threshold potential typically ranges around -55 mV to -50 mV, varying between different neuron types. When the membrane potential reaches this critical point, voltage-gated sodium channels open, allowing sodium ions to flood into the cell. This influx of positively charged ions leads to further depolarization, creating a self-amplifying process that results in a full action potential. The ability of a neuron to reach threshold potential influences its excitability and responsiveness to stimuli.
The importance of threshold potential cannot be overstated. It serves as a gatekeeper for neuronal firing. If a stimulus is not sufficient to reach this threshold, the neuron does not fire, which ensures that signals are transmitted efficiently and only when necessary. This selective firing is crucial for maintaining the fidelity of neural circuits and preventing random noise in neuronal activity.
Factors Influencing Threshold Levels
Several factors can influence the threshold levels of neurons, affecting their excitability and overall function. Some key factors include:
- Ion Concentration: The balance of sodium, potassium, calcium, and chloride ions across the neuronal membrane affects the resting potential and can thus shift the threshold limit.
- Membrane Properties: The lipid composition and state of the axonal membrane, particularly the presence of myelin, can alter how easily the membrane can depolarize and thus influence the threshold.
- Age and Health of the Neuron: Neuronal health, which can be affected by factors such as age, injury, or disease, also plays a role in determining the excitability of the neuron and its threshold potential.
- Neurotransmitter Interactions: The binding of neurotransmitters to receptor sites can change the excitability of a neuron, often either raising or lowering its threshold potential depending on the type of neurotransmitter and receptor involved.
Understanding threshold potential and its influences is crucial for grasping how neurons communicate and how various neurological disorders can arise when this process is disrupted.
In summary, threshold potential is not just a simple measurement; it is pivotal for the functioning of neuronal systems, impacting everything from basic reflexes to complex cognitive processes.
Propagation of Action Potential
The propagation of action potential is a crucial component in understanding neuronal signaling. This process ensures that electrical impulses can effectively travel across long distances within the nervous system. Without proper propagation, communication between neurons would be severely hindered, affecting overall neural function. The mechanisms involved in propagation are essential for both physiological function and pathological events, making this a pertinent area of study in neuroscience.
Saltatory Conduction
Saltatory conduction is a highly efficient form of action potential propagation that occurs in myelinated neurons. In this process, the action potential jumps between nodes of Ranvier. These nodes are small gaps in the myelin sheath that allow for the concentrated presence of voltage-gated sodium channels. The rapid depolarization and repolarization at these nodes drastically increase the speed of conduction.
Some benefits of saltatory conduction include:
- Increased speed: The action potential can travel much faster than it would in unmyelinated fibers.
- Energy efficiency: As sodium ions need to be pumped out of the neuron less frequently, the neuron's energy consumption is reduced.
- Enhanced functionality: Faster transmission allows for more complex physiological responses.
Continuous Conduction
In contrast to saltatory conduction, continuous conduction occurs in unmyelinated fibers. Here, the entire neuronal membrane depolarizes in a wave-like manner. The potential propagates through the entire length of the axon without interruption. This is a slower process than saltatory conduction, but it is still effective in transmitting signals where speed is not as critical, such as in smaller, peripheral nerves.
Key characteristics of continuous conduction are:
- Uniform propagation: Without myelin, each segment of the axon initiates an action potential, leading to a slower overall signal transmission.
- Consistent ionic movement: The entire membrane participates in propagating the action potential, allowing for reliable signaling over shorter distances.
Factors Affecting Conduction Velocity
Several factors can influence the conduction velocity of an action potential in neurons. Understanding these factors provides insight into how variations in conduction can affect neural function.
Factors include:
- Axon diameter: Larger axons typically conduct impulses faster than smaller ones due to reduced resistance.
- Presence of myelin: Myelination significantly enhances conduction speed by enabling saltatory conduction.
- Temperature: Higher temperatures usually increase conduction velocity, while lower temperatures may slow it down.
- Ion concentration: Changes in ion concentrations, particularly sodium and potassium, can impact the rate at which action potentials are generated and propagated.
The efficiency and speed of action potential propagation are fundamental to rapid communication in the nervous system. Understanding these dynamics can help address various neurological disorders that impair neuronal signaling.
Role of Neurotransmitters
Neurotransmitters play a crucial role in the functioning of neurons, facilitating communication between nerve cells and influencing various neurological processes. These chemical messengers are essential for transmitting signals across synapses, which is vital for the proper functioning of the nervous system. Understanding the mechanisms and effects of neurotransmitters helps illuminate their significance in both normal physiology and in the context of neurological disorders.
Neurotransmitters have various functions, including the modulation of mood, cognition, and motor control. They influence many brain activities through excitatory and inhibitory effects on target neurons. This balance is essential for maintaining healthy brain function. The interplay of different neurotransmitters can affect everything from simple reflexes to complex behaviors and emotions.
Mechanisms of Neurotransmitter Release
The release of neurotransmitters occurs through a well-orchestrated process that ensures precise signaling between neurons. When an action potential arrives at the presynaptic terminal, it causes voltage-gated calcium channels to open. Calcium ions then flow into the neuron, triggering synaptic vesicles filled with neurotransmitters to fuse with the membrane and release their contents into the synaptic cleft.
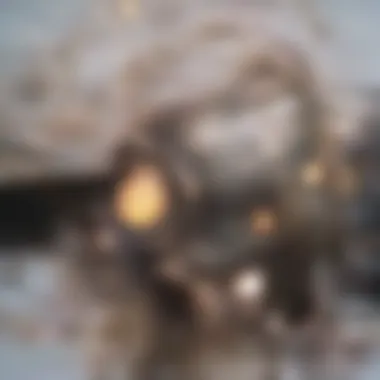
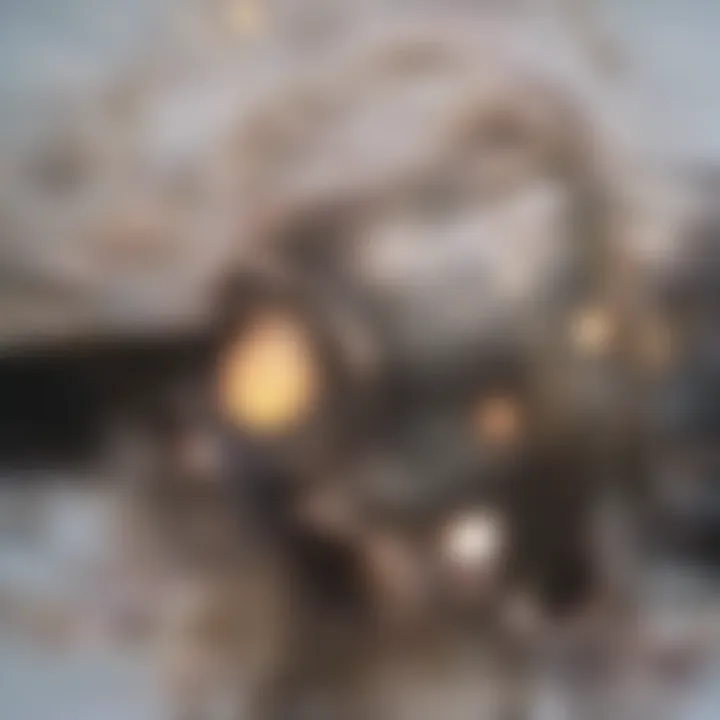
This process can be detailed in several steps:
- Action potential arrival: The action potential travels down the axon, reaching the synaptic terminal.
- Calcium influx: The opening of voltage-gated calcium channels allows calcium ions to enter the neuron.
- Vesicle fusion: Calcium binding causes synaptic vesicles to move towards the membrane and merge with it, releasing neurotransmitters into the synaptic gap.
- Diffusion: Neurotransmitters diffuse across the synaptic cleft and bind to specific receptors on the postsynaptic neuron.
This sequence is essential for the propagation of signals in the nervous system and highlights the importance of neurotransmitter release in neuronal communication.
Receptors and Signal Transduction
After neurotransmitters are released, they bind to specific receptors on the postsynaptic neuron. This binding initiates signal transduction, which converts the external chemical signal into a functional response within the cell. Neurotransmitter receptors can be broadly categorized into two types: ionotropic and metabotropic receptors.
- Ionotropic receptors: These receptors are ligand-gated ion channels that open in response to neurotransmitter binding. The opening allows specific ions, such as sodium, potassium, or chloride, to flow into or out of the cell, resulting in rapid changes in membrane potential.
- Metabotropic receptors: These receptors are G-protein coupled receptors that indirectly influence neuronal activity through second messengers. The activation of these receptors leads to a cascade of intracellular effects that can alter gene expression and modify neuron function over a longer duration than ionotropic receptors.
Both receptor types play critical roles in synaptic transmission, and their interaction with neurotransmitters is fundamental in processing and integrating signals in the nervous system.
Neurotransmitters are the keys to unlocking the complex communication network of the brain, influencing everything from simple reflexes to complex behaviors.
Pathological Implications
The study of action potential dysfunction is crucial in understanding various neurological disorders. When the mechanisms of action potential fail, the consequences can be severe, affecting neuronal communication and leading to a range of pathologies. Disorders resulting from abnormal action potentials distinctly illustrate how essential these electrical signals are for normal brain function. This section examines some of these disorders and the ongoing research that aims to elucidate and treat them.
Disorders Related to Action Potential Dysfunction
Several neurological conditions stem from disruptions in action potential generation and propagation. These disorders can impact both peripheral and central nervous systems. Key examples include:
- Epilepsy: Characterized by irregular electrical discharges in neurons, leading to seizures. The disorder is often due to imbalances in excitatory and inhibitory neurotransmitter systems, affecting action potentials.
- Multiple Sclerosis: This autoimmune disease damages the myelin sheath, impeding the conduction of action potentials. As a result, patients may experience symptoms like impaired coordination and fatigue.
- Myasthenia Gravis: In this condition, antibodies destroy or block nicotinic acetylcholine receptors at the neuromuscular junction, disrupting the signaling process. This leads to muscle weakness and fatigue, affecting motor control.
- Congenital Long QT Syndrome: Genetic mutations in cardiac ion channels lead to prolonged action potentials within the heart. This can result in serious arrhythmias.
Each of these disorders highlights the necessity of properly functioning action potentials for maintaining neuronal health and overall system balance.
Research Developments in Neurological Disorders
Ongoing research is crucial for understanding the mechanisms behind action potential dysfunction and developing potential treatments. Some notable advancements include:
- Gene Therapy: Researchers are exploring methods to correct genetic mutations that lead to ion channel dysfunctions. This approach holds promise for conditions like congenital long QT syndrome, where therapies could restore normal action potential rhythms.
- Antibody Treatments: Efforts are underway to create therapies targeting autoimmune responses seen in multiple sclerosis and myasthenia gravis. By modulating the immune system, it may be possible to enhance the integrity of action potentials.
- Neuroprotective Strategies: Scientists are investigating compounds that protect neurons from damage during seizures or other pathological states. This could help preserve action potential integrity in conditions like epilepsy.
- Advanced Imaging Techniques: New imaging methods enable researchers to observe action potential dynamics in real time. This is beneficial for understanding disease progression and response to therapies in live models.
Understanding the implications of action potential dysfunction reveals the complexities of neuronal communication, significantly shaping how we approach treatment and management of these disorders.
Current Research Trends
Current research trends in neuronal action potentials are crucial for advancing our understanding of neuronal function and dysfunction. With the growing interest in neuroscience, researchers are uncovering new methods and insights that change how we look at neuronal signaling. The recent nuances in this area hold significant implications for medical science, especially concerning neurodegenerative diseases and psychiatric disorders.
Innovative Studies in Neuronal Physiology
Innovative studies in neuronal physiology focus on the dynamic processes that underlie action potential generation and propagation. Researchers are utilizing cutting-edge techniques such as optogenetics and advanced imaging to visualize and manipulate neuronal activity in real time. This approach provides unprecedented insights into how ion channel activity correlates with neuronal firing patterns.
- Optogenetics: This technique allows researchers to control specific neurons using light, enabling the elucidation of the roles of various neurotransmitters in action potential dynamics.
- Imaging Technologies: Advanced imaging methods, such as two-photon microscopy, provide a way to observe neuronal circuits in living organisms, revealing how these networks interact during action potential firing.
Such studies have prompted a reevaluation of existing models of neuronal behavior, suggesting that previous assumptions about uniformity in neuronal activity may not hold true under diverse physiological conditions. Research also highlights the variability in action potential characteristics across different neuron types, indicating a more complex interplay than previously typical.
Advances in Neurotechnological Applications
Advancements in neurotechnological applications are vital for translating findings from fundamental research into practical tools that can aid in understanding and treating neurological disorders. One notable area of development includes brain-computer interfaces (BCIs), which leverage knowledge of neuronal action potentials to establish real-time communication pathways between the brain and external devices. These devices have great potential in rehabilitation for individuals with severe mobility impairments.
Furthermore, the integration of artificial intelligence in analyzing neuronal data is transforming the landscape of neuroscience research. AI systems can process large datasets generated from neuronal activity, uncovering patterns that may not be immediately apparent to human researchers. This can lead to the identification of new therapeutic targets for diseases like Alzheimer's and Parkinson's.
The implications reach beyond just understanding neuronal activity. They extend to practical applications in mental health, informing the development of interventions tailored to conditions such as depression, where imbalances in neuronal signaling could be restored through innovative treatments.
Researchers are also exploring the business side of neurotechnology. Startups are emerging with novel applications focused on increasing the capacity for neural repair and regeneration, highlighting the economic and societal interest in enhancing our neurological health.
Recent studies are paving the way for potentially life-changing applications, bringing hope for better management of neurological disorders through technological innovation.
Epilogue
The conclusion of this article emphasizes the integral role of action potential within the realms of neuroscience and its broader implications for understanding neuronal function. It is essential to recognize that action potentials are not mere electrical impulses but are pivotal in propagating signals across neuronal networks. Important elements of this discussion include the mechanisms of action potentials, their phases, and how variations in their properties can lead to significant physiological changes or disorders.
Summary of Key Points
In summarizing the key points highlighted throughout the article, it is clear that:
- Definition of Action Potential: Action potentials are rapid, transient changes in membrane potential that allow neurons to communicate.
- Phases Explored: The phases of action potential—depolarization, repolarization, and hyperpolarization—were detailed, illustrating how ion movement influences neuronal signaling.
- Propagation Mechanisms: The differences between saltatory and continuous conduction were discussed, emphasizing how myelination speeds up signal transmission.
- Role of Neurotransmitters: The significance of neurotransmitters in synaptic transmission following action potentials was addressed.
- Pathological Implications: Disorders such as epilepsy and multiple sclerosis can stem from dysfunctional action potentials, highlighting the need for further research.
Future Directions in Neuronal Research
Future directions in neuronal research must take into account the evolving understanding of action potentials. Areas worthy of exploration include:
- Innovative Techniques in Imaging: Advancements in imaging technologies can facilitate real-time observation of action potentials within living organisms, allowing for detailed analyses of neuronal dynamics.
- Investigations at the Molecular Level: Research into the molecular mechanisms governing ion channels and pumps can lead to better understanding of their roles in health and disease.
- Therapeutic Approaches: With a clearer comprehension of action potentials, researchers may develop targeted therapies for neurological disorders linked to their dysfunction, potentially improving treatment outcomes.
- Neuroinformatics and Modeling: Utilizing computational models to simulate neuronal behavior may provide deeper insights into how action potentials work within larger neural networks.
Understanding action potentials is fundamental not just in the study of neurology, but in applying these insights to clinical practices and therapeutic innovations. The future of this research remains dynamic and holds promise for significant advancements.