Amino Acid to Nucleotide Sequence Conversion Insights
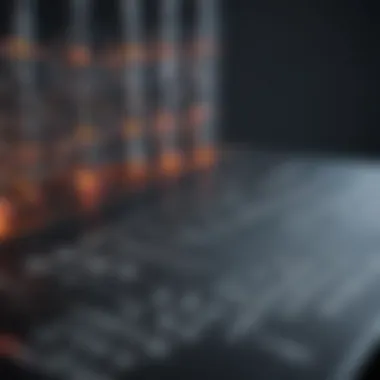
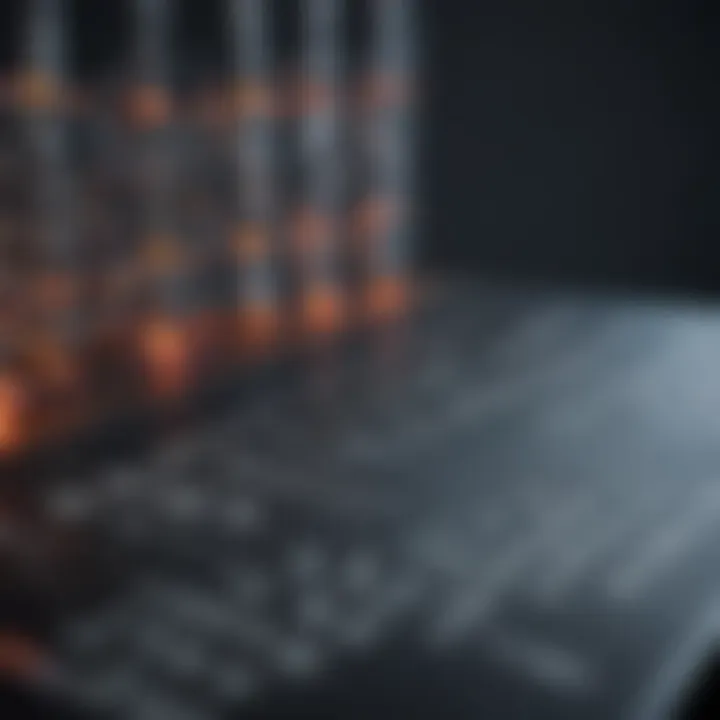
Intro
The conversion of amino acids to nucleotide sequences is no simple task; it’s a journey through the realm of molecular biology that brings together various elements of genetics and biochemistry. In a nutshell, amino acids are the building blocks of proteins, while nucleotides form the backbone of DNA and RNA. Understanding how these two sets of molecules interact is essential for many fields, including genetic engineering, drug development, and synthetic biology.
This article aims to unpack this intricate process, shedding light on the mechanisms at play. From the basic understanding of the genetic code to the specific methodologies used for conversion, we aim to give a full picture of how these molecular languages translate into one another. The implications of this conversion extend beyond pure academic interest—they are at the forefront of biotechnological innovations and applications.
Let’s peel back the layers and get into the nuances of this fascinating topic.
Understanding Amino Acids
Amino acids are often referred to as the building blocks of proteins, and their importance cannot be overstated when delving into the intricacies of molecular biology. Without a firm grasp of amino acids, one might as well be trying to read a book written in a foreign language without knowing the alphabet. They play crucial roles in numerous biological processes, including the synthesis of proteins, which are essential for the structure, function, and regulation of the body's tissues and organs.
Their chemical structure characterizes amino acids, each possessing an amino group, a carboxyl group, and a distinctive side chain. This side chain influences not just the physical and chemical properties of the amino acid itself but also how it interacts with others, forming the elaborate networks that are proteins. Consider, for instance, how some amino acids can form hydrogen bonds, while others can create hydrophobic cores. This diversity in structure essentially provides the tools needed for creating the vast array of proteins found in nature.
The classification of amino acids further enhances our understanding of their functions. By dividing them into categories such as essential versus non-essential, polar versus non-polar, and charged versus uncharged, we can comprehend their varying roles in biological systems and their implications when discussing protein synthesis and function. This article will provide a close examination of these classifications, the resulting properties, and how these translate during the process of converting amino acid sequences into nucleotide sequences.
"All proteins are made from the same 20 amino acids, but the specific sequence of these amino acids can vary, leading to incomprehensibly vast diversity in protein functions."
The importance of understanding amino acids in the context of this article is not merely academic; it has practical implications as well, particularly in biotechnology and genetics. Whether it is designing a new protein in synthetic biology or understanding gene expression and regulation, a foundational knowledge of amino acids is essential. In summary, this exploration of amino acids will set the stage for revealing their role in the journey to nucleotide sequences, enabling a better comprehension of the genetic blueprint of life.
Exploring Nucleotides
Nucleotides are the building blocks of nucleic acids, and understanding their structure and function is a critical component in the study of molecular biology. The conversion of amino acid sequences into nucleotide sequences cannot be fully appreciated without a grasp of what nucleotides are and how they operate within the cellular machinery. This topic forms a bridge between protein synthesis and genetic encoding, highlighting how these tiny molecules play immense roles in the greater context of life itself.
Nucleotides serve multiple functions beyond just being components of DNA and RNA; they are also vital in energy transfer, signaling, and metabolism. Their intricate structure and varying roles underscore their importance. Delving into the structural components and differences between nucleotides in DNA and RNA offers a foundational understanding necessary for comprehending how amino acid sequences are translated into genetic information.
Structural Components of Nucleotides
To dive deeper into nucleotides, one must first dissect their structure, which comprises three core components: a nitrogenous base, a five-carbon sugar, and one or more phosphate groups.
- Nitrogenous Base: This part varies between different nucleotides. There are two main types:
- Five-carbon Sugar: This is either ribose or deoxyribose. Ribose is found in RNA, while deoxyribose, which lacks one oxygen atom, is found in DNA.
- Phosphate Group: Nucleotides can have one, two, or three phosphate groups attached, which is crucial in the formation of nucleic acids. The presence of these groups impacts their energy levels and how they connect within chains.
- Purines (adenine, guanine) - double-ring structures.
- Pyrimidines (cytosine, thymine, uracil) - single-ring structures.
These components come together to form a nucleotide, where the phosphate group of one nucleotide bonds with the sugar of another. Such connections create phosphodiester bonds, leading to the formation of long chains that compose the nucleic acids.
Nucleotides in DNA vs RNA
Nucleotides play different roles in DNA and RNA, which are fundamental in the process of translating amino acids into nucleic sequences.
- DNA Nucleotides:
- RNA Nucleotides:
- Composed of deoxyribose sugar.
- Contains the bases adenine, guanine, cytosine, and thymine.
- Form double-stranded helical structures essential for genetic storage.
- Made up of ribose sugar.
- Contains bases adenine, guanine, cytosine, and uracil (instead of thymine).
- Typically single-stranded and involved primarily in protein synthesis and transcription processes.
The differences between these nucleotides are not mere trivia; they influence their functionality considerably. For instance, the stability of DNA allows it to serve as a long-term repository for genetic information, while RNA's more versatile structure facilitates its roles in the cellular processes of translation.
Understanding these distinctions is crucial when considering how the genetic code is represented and eventually translated into the amino acid sequences that dictate the diversity of proteins in living organisms.
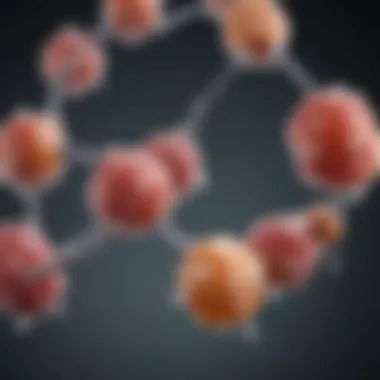
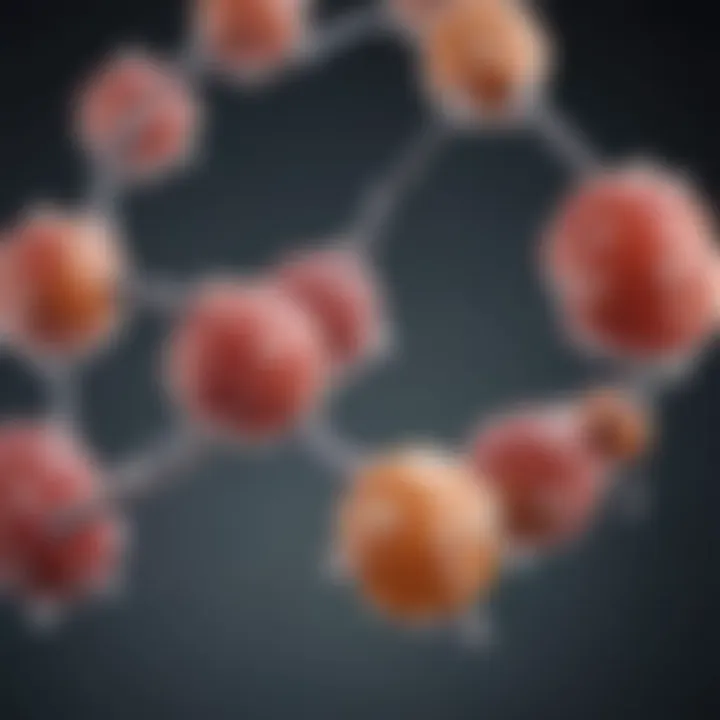
The Genetic Code
The genetic code serves as the foundation for the relationship between amino acids and nucleotide sequences. Each triplet of nucleotides, known as a codon, corresponds to a specific amino acid or serves as a signal for the beginning or end of protein synthesis. This appeals not only to molecular biologists but also to those in biotechnology and related fields, due to the implications of the genetic code in various biotechnological applications.
Understanding the genetic code is crucial for anyone who ventures into protein synthesis. First and foremost, it’s essential to grasp how codons dictate which amino acids are assembled to form proteins, which are vital for nearly every process within living organisms. Furthermore, the nuances of codon usage can greatly impact the efficiency and accuracy of the translation process.
"The genetic code is universal, but how it’s interpreted can change from one organism to another."
Exploring the structure and function of codons opens the door to understanding their roles in a broader context of genetic expression. While there are only 20 standard amino acids, the code contains 64 codons, accounting for redundancy. This redundancy is not a superfluous detail; it plays a significant role in how proteins are translated in different biological contexts .
Codons and Their Function
Codons are integral to the process of translation. Made up of three nucleotides, these specific sequences instruct the ribosome during protein synthesis. For instance, the codon AUG is often designated as the start codon, indicating the point at which translation commences. The subsequent codons dictate the addition of distinct amino acids to the growing polypeptide chain, thus shaping the protein's final structure and function.
Particularly notable is the distinction that different codons can specify the same amino acid. For example, both GCU and GCC designate Alanine. This phenomenon plays into the resilience of biological systems, as mutations occurring in the genetic code do not always result in alterations of the protein formed, which can be critical for organismal survival and adaptability.
- Unique codon sequences: Different species may exhibit preferences for certain codons over others, known as codon bias. This is crucial for optimizing gene expression in systems such as synthetic biology.
- Start and stop codons: Codons are responsible for signaling the beginning and end of a protein, allowing ribosomes to translate genetic information accurately.
Redundancy and Universality
The redundancy of the genetic code is a fascinating aspect that holds deep significance in the life sciences. While there are numerous codons, only a limited number correspond to specific amino acids. This redundancy ensures that some mutations or variability in the nucleotide sequences can occur without affecting the resulting protein.
Redundancy provides a buffer against potential errors in the translation process, allowing organisms to maintain stability despite genetic fluctuations. Importantly, though there are variations in codon utilization across different organisms, the core essence of the genetic code remains consistent.
- Universal Language of Life: The universality of the genetic code implies that genes from one organism can be expressed in another, which is pivotal for genetic engineering and synthetic biology.
- Evolutionary Significance: The shared genetic code among diverse life forms hints at a common ancestry, emphasizing the evolutionary links that tie all life together.
- Applications in Biotechnology: Understanding this redundancy is fundamental to manipulating DNA sequences for biotechnology applications, such as gene therapy or the production of recombinant proteins.
The genetic code is indeed a marvel of molecular biology, possessing layers of complexity that reveal how life translates information at a fundamental level. As advancements in bioinformatics continue to enhance our understanding, the intricacies of codon usage, redundancy, and universality will prove indispensable to the future of research and application within both basic and applied biology.
Conversion Mechanism: Amino Acid to Nucleotide
The transformation of amino acid sequences into their corresponding nucleotide sequences lies at the heart of molecular biology. This conversion process is not merely an academic exercise; it is fundamental to understanding how genes encode proteins, which in turn dictate the function of every living organism. The correct translation hinges on a nuanced grasp of both the genetic code and the mechanics of protein synthesis.
Investigating this conversion process reveals several key benefits. First, it opens avenues for genetic engineering and biotechnology, allowing scientists to manipulate the code of life. Second, comprehending this mechanism is crucial for interpreting variations in protein function, which can lead to innovations in medicine and science. Hence, understanding the specifics behind this mechanism can set the stage for advancements in numerous fields, from agriculture to pharmaceuticals.
Translation Process Overview
Understanding the process of translation is essential to fully grasp the conversion from amino acids to nucleotide sequences. This can be boiled down to three main phases: Initiation, Elongation, and Termination. Each phase serves a unique function in ensuring the accurate synthesis of proteins from amino acids.
Initiation
The initiation phase marks the starting point of translation, where the ribosome assembles around the messenger RNA (mRNA). Here, the first amino acid, usually methionine, is brought into position. This phase is particularly critical as it sets the reading frame for the rest of the process. One defining characteristic of initiation is its dependency on the sequence of nucleotides in mRNA. It’s essential because a misstep here can lead to significant errors, affecting the entire protein. This phase is often seen as a beneficial aspect of translation because it ensures that ribosomes are ready to translate the genetic code accurately. However, complications may arise from variations in initiator sequences, leading to challenges in translating certain genes efficiently.
Elongation
Following initiation, the elongation phase tends to be where the action really heats up. This is where the ribosome moves along the mRNA, adding amino acids one by one to the growing polypeptide chain. A key characteristic of elongation is the rapid addition of amino acids, which can occur at a remarkable speed. The sequence of codons in the mRNA directly dictates this process, making it a vital component in ensuring that the resulting protein is constructed correctly. One unique feature of elongation is the role of transfer RNA (tRNA), which brings amino acids into the ribosome according to the codons. While this phase is generally viewed positively due to its efficiency, any mistakes during elongation can lead to misfolded proteins, which have the potential to be dysfunctional or harmful.
Termination
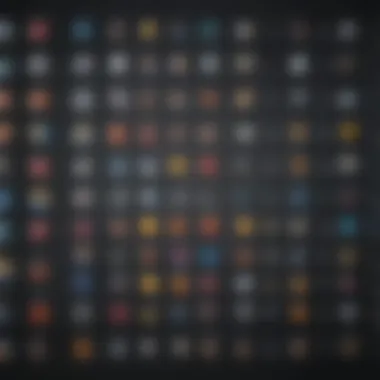
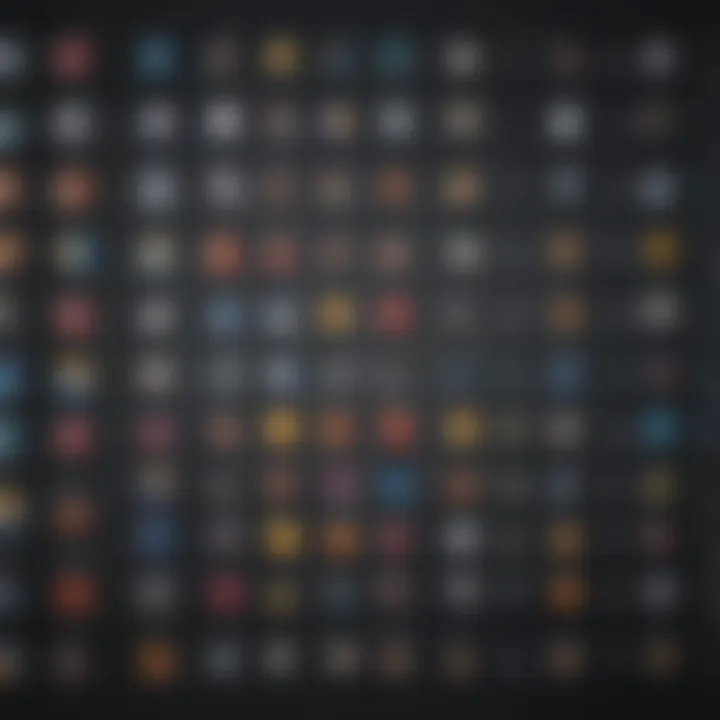
Termination is the finale of the translation process, signaling the end of protein synthesis. It occurs when a stop codon on the mRNA is reached, signaling that the complete protein has been assembled. One notable characteristic of termination is the role of release factors, which help disassemble the ribosomal complex. This phase is beneficial because it not only ensures that the entire protein is synthesized accurately but also facilitates the recycling of the ribosomal machinery for future translation events. However, improper termination can result in incomplete or nonfunctional proteins, leading to further complications in cellular functions.
Codon Mapping Techniques
Mapping codons to their respective amino acids is crucial for accurately converting sequences from one format to another. Several techniques are employed in this context, including:
- Graphical Codon Charts: These provide visual representations of which codons correspond to which amino acids.
- Codon Usage Tables: By showing the frequency of each codon in different organisms, such tables can guide engineers in selecting the most efficient codons for synthetic biology applications.
- Software Tools: Various bioinformatics software can facilitate the mapping and conversion process, streamlining the workflow for researchers.
Software and Tools for Conversion
The advent of technology has birthed numerous software tools designed to assist in the conversion of amino acid sequences to nucleotide sequences. These tools often feature user-friendly interfaces that allow for quick input of sequences and rapid output of relevant nucleotide translations. Popular software options include:
- ExPASy: This platform provides a range of tools for translating sequences and conducting bioinformatics analysis.
- SnapGene: Well known in synthetic biology, it facilitates easy manipulation and visualization of sequences.
- Geneious: A comprehensive solution that offers various functions for DNA analysis, including translation capabilities.
Each of these tools provides distinct advantages, enabling researchers to perform more precise and rapid sequence conversions. However, one must be cautious of the discrepancies that might arise from using different codon preferences or organism-specific variations in translation, which can result in inaccuracies.
In summarizing the conversion mechanism, it becomes clear that the journey from amino acids to nucleotides is anything but straightforward. Understanding every phase — initiation, elongation, termination — not only enriches our comprehension of protein synthesis but also enhances our ability to manipulate these processes for scientific advancement.
Factors Impacting Conversion Accuracy
The conversion from amino acid sequences to nucleotide sequences isn't just a simple table lookup or a straightforward calculation. It involves several factors that can influence the accuracy of this conversion profoundly. Understanding these factors can be essential not only for researchers and biologists but also for anyone involved in genetic engineering and synthetic biology. When errors creep in during conversions, they can lead to significant implications in protein synthesis and functionality, affecting everything from basic research to medical applications.
Codon Preference Bias
Codon preference, often termed “codon usage bias,” relates to how certain codons are favored over others for a specific amino acid in a given organism. For instance, the same amino acid can be encoded by multiple codons, but not all organisms utilize these codons equally. Here are some key points regarding codon preference bias:
- Genomic Context: Some regions of a genome might display specific codon usages. This variability can stem from evolutionary pressures, leading one organism to prefer a particular codon over its counterparts.
- Efficiency in Translation: The translation apparatus of a cell evidently prefers certain codons, which could enhance the efficiency of protein synthesis. High-abundance tRNAs corresponding to favored codons can lead to faster and more accurate translation.
- Impacts on Protein Folding: When codon bias is ignored, this could lead to proteins that fold incorrectly or form aggregates, creating functional hurdles.
A study might show, for example, that Escherichia coli prefers the codon "GAA" for glutamic acid, while Yeast may favor "GAG". Understanding these nuances can offer insights into optimal designs for synthetic genes that may need to be produced in different host organisms.
Organism-Specific Factors
Organisms are not just mere hosts for genes; they each have their distinct biological roles and mechanisms affecting how genetic information is processed and interpreted. When converting amino acids to nucleotides, several organism-specific considerations can arise, such as:
- Genetic Background: The genetic architecture, including the presence of alternative splicing or the presence of specific regulatory elements, can significantly affect conversion accuracy.
- Introns and Exons: In eukaryotic organisms, introns can complicate the conversion process, as these non-coding regions must be spliced out before the mature mRNA is translated into proteins.
- Environmental Factors: Conditions such as temperature and pH that influence the living organisms can further impact protein expression, thereby affecting the nucleotide sequence needed for optimal expression and synthesis.
"Understanding organism-specific factors is crucial because not every gene is treated the same across different species, and that can lead to variance in gene expression."
As researchers decode and synthesize genes with consideration to these specific factors, the longevity and functionality of produced proteins stand to gain considerably. The careful consideration of codon preferences and organism-specific quirks creates both a challenge and an opportunity to ground-breaking advancements in biotechnology.
Applications in Biotechnology
The translation from amino acid sequences to nucleotide sequences is a cornerstone of molecular biology, providing the theoretical framework for numerous innovative applications in biotechnology. At its core, this conversion process empowers scientists to manipulate genetic material in ways that were once considered unimaginable. Understanding this intricate relationship opens the doors to various biotechnological advancements that impact fields ranging from medicine to agriculture.
One of the most significant benefits of translating amino acids to nucleotides lies in synthetic biology. This field employs engineering principles to construct new biological parts, devices, and systems. For instance, the ability to design proteins with specific functions can only be achieved by understanding how their sequences correspond to DNA. Synthetic biology enables the creation of custom enzymes tailored for industrial processes, enhancing efficiency and reducing costs. Moreover, it allows for bio-based production systems, which utilize living organisms as factories to generate valuable compounds.
Conversely, the implications of genetic engineering stretch beyond the lab, influencing public health and food security. The capability to alter nucleotide sequences in crops can lead to genetically modified organisms (GMOs) that are more resistant to diseases or environmental stresses. This is crucial as global populations continue to grow, necessitating higher agricultural yields while minimizing the use of harmful chemicals.
"By understanding and applying amino acid to nucleotide conversion, we pave the way for biotechnology that can solve some of humanity's most pressing challenges."
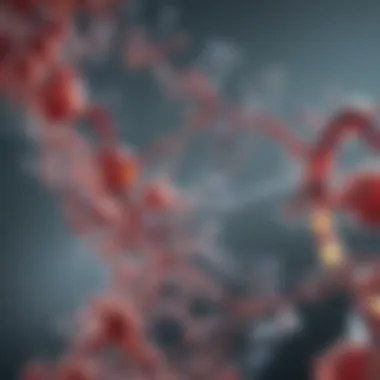
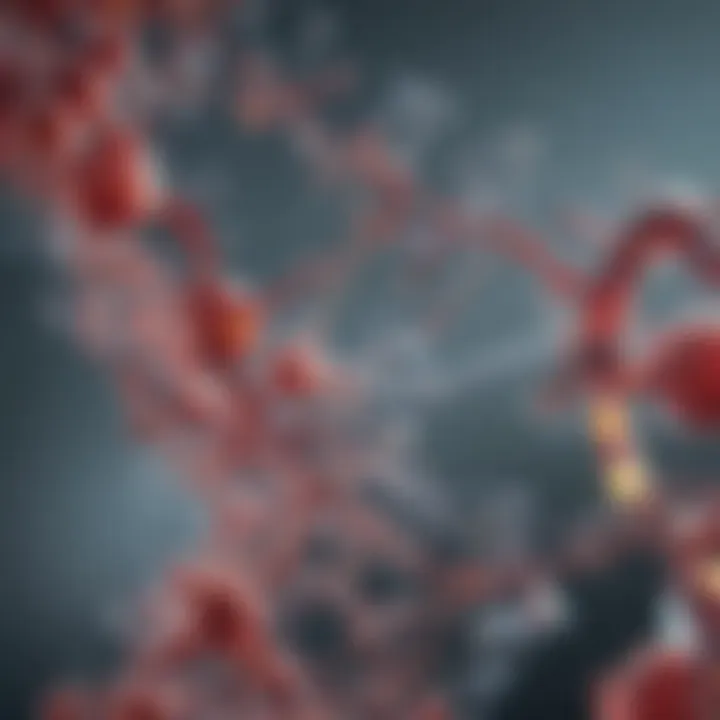
The intertwined nature of protein synthesis and genetic manipulation forms a robust foundation for gene editing techniques, which are paramount in modern biotechnology. Edits made at the DNA level, such as through sticking in novel sequences or altering existing ones, have profound implications for research and application purposes.
In summary, the relevance of amino acid to nucleotide sequence conversion in biotechnology underscores the necessity for ongoing research and development. By harnessing the knowledge of translation mechanisms, scientists can drive innovations that address both local and global issues, making strides in synthetic biology and beyond. The potential applications are not merely abstract concepts; they are vital tools in creating a sustainable future.
Challenges in Amino Acid to Nucleotide Conversion
The transformation of amino acid sequences into nucleotide sequences is a complex affair, filled with nuances that can trip up even seasoned molecular biologists. Understanding the challenges faced in this process is essential, not just for grasping basic concepts but also for appreciating the broader significance of accuracy in genetic engineering and synthetic biology.
Errors in the translation process can have cascading effects on an organism's functionality, affecting everything from basic cellular processes to the development of disease. Identifying and addressing these challenges provides insight into the very foundation of genetic expression. Here are key elements to consider:
- Translation Errors: These errors can arise from mismatched codons during the translation phase, leading to incorrect amino acid incorporation into proteins.
- Codon Preference: Not all organisms utilize codons equally. Recognizing codon bias is paramount in synthetic designs to ensure that the desired protein is expressed efficiently.
- Environmental Factors: Variables such as temperature and ion concentrations can influence enzyme activity involved in translation, introducing further inconsistencies in the conversion process.
Clearly, navigating these obstacles is not just an academic exercise; it has real-world implications that extend into biotechnology and therapeutic developments.
Errors in Translation
Translation errors can emerge from several layers of complexity, especially when a sequence translates incorrectly due to misread codons. Such errors may occur because of:
- mRNA changes: Mutations in the mRNA sequence can lead to faulty codon assignments.
- Ribosome activity: The ribosome may encounter a blockage, causing it to skip over nucleotides, ultimately resulting in incomplete protein synthesis.
- Aminoacyl-tRNA mischarging: If the wrong amino acid is attached to a tRNA molecule, it can create a cascade of mistaken translations that compromises protein integrity.
These mistakes can lead to proteins with altered structures and functions, and in some cases, can even result in outright non-functionality.
Implications for Protein Function
The implications of translation errors cannot be overstated. When proteins do not fold correctly or incorporate the wrong amino acids, several consequences may arise:
- Loss of Function: Proteins may lose their capability to perform biological tasks if their structures are altered significantly.
- Diseased States: Mutations in protein sequences are linked to various diseases, including cystic fibrosis and sickle cell anemia, highlighting the importance of accuracy in translation.
- Cellular Control: Proteins play pivotal roles in regulating cellular processes. An incorrectly translated protein could disrupt these control points, leading to catastrophic cellular behavior.
"Every tiny shift in the amino acid sequence could potentially lead to a grand malfunction in cellular behavior."
Addressing these challenges involves not merely recognizing where mistakes can happen, but also developing methods for mitigating them. As biotechnology advances, tools and software that streamline accurate conversion from amino acid to nucleotide sequences will become even more vital.
Future Perspectives
The shifting landscape of molecular biology is undeniably exciting, particularly when it comes to the conversion of amino acid sequences into nucleotide sequences. As researchers dive deeper into this intricate process, it’s crucial to understand how advancements in this field might evolve and influence both practical application and theoretical exploration. This section features some notable trends and upcoming developments.
Advances in Bioinformatics
Bioinformatics is transforming the way we perceive amino acid to nucleotide conversion. The intricate process demands robust computational tools capable of handling vast amounts of biological data. Integrating machine learning algorithms into bioinformatics can accelerate the identification of patterns and relationships between amino acids and their corresponding nucleotides, enhancing the accuracy of conversions.
Benefits include:
- Precision: Algorithms can be trained to predict amino acid sequences more accurately by studying codon usage bias in varied organisms.
- Speed: With the increasing power of computational resources, conversions that once took days can be accomplished in hours, if not minutes.
- Customization: Tools can be designed specifically for certain organisms or experimental conditions, providing tailored approaches dependent on research needs.
As bioinformatics continues to grow, its intersection with amino acid to nucleotide conversion will likely yield novel methodologies, refining the translational processes that form the backbone of genetic research and applications.
Emerging Technologies in Protein Engineering
The horizon of protein engineering is broadening with groundbreaking technologies paving the way for a more refined understanding and manipulation of amino acids and nucleotides. Biotechnological advancements encompass a range of techniques that potentiate innovation in how proteins are designed and utilized.
An interesting trend in this space is the application of CRISPR technology. This revolutionary editing technique allows for precise modifications at the genetic level, which means researchers can potentially alter the nucleotide sequences directly to counteract or enhance protein functions. For instance, it can be used to correct mutations in genes that lead to disease, providing direct applications for human health.
Moreover, TALEN (Transcription Activator-Like Effector Nucleases) also holds great promise. Much like CRISPR, TALENs provide targeted genetically modified organisms by recognizing and cleaving specific sequences, allowing precise control. As these technologies enhance the efficiency with which proteins are engineered, they directly impact our ability to translate between amino acid sequences and nucleotide sequences.
The expansion in protein engineering techniques not only contributes to a deeper understanding of biological processes but also opens doors to innovative applications in medicine, agriculture, and beyond.
In summary, as we look toward the future, both bioinformatics and emerging protein engineering technologies are poised to significantly impact the processes involved in amino acid to nucleotide conversion. The capacity for rapid advancements and novel applications means that staying updated with the latest research and technological innovations will be imperative for students, researchers, and professionals engaged in this dynamic field.